1 Introduction
How do you usually know whether you are conscious? The only way is to have subjective experience with some kind of content, no matter whether a crisp or a vague one. Without entering philosophical debates, we take this to mean that the contents and state of consciousness are intimately related. In some states contents are crisp, vivid, stably present and rich (alert wakefulness), in some other states contents are vivid, but less coherent (e.g. dreams, psychedelic hallucinations), in yet other states contents are less vivid, instable, relatively poor (e.g., sleep or hypnagogic state), in some states they are sporadic, minimally expressed and fully out of deliberate control (minimally conscious state), and in some brain states conscious contents are absent (dreamless sleep, coma). In short, the characteristics of the contents of consciousness depend on the state of consciousness.
There is a lot of evidence to show that the contents of consciousness are “encoded” in the cortex. Neurobiology and science of clinical anesthesia also have a lot of converging evidence that workings of subcortical structures, especially certain parts of the thalamus are also necessary for consciousness. A theory of consciousness thus has to try to find a way to explain consciousness by simultaneously invoking both cortical and subcortical mechanisms. More than 20 years ago one of the authors of this paper described a theory based on interaction of the nonspecific thalamus and cortical content-encoding neurons so as to explain a number of conscious-perception phenomena (Bachmann, 1998). The model of conscious perception was based on excitatory post-synaptic potentials brought about by pre-synaptic afferents from the specific, content-carrying system and afferents from non-specific modulation system. According to the model (Bachmann, 1994), for the content to be consciously experienced, the cortical content-carrying neurons had to be modulated by the afferents from the non-specific subcortical neurons. The issue of the state of consciousness remained out of the scope. Over these years, however, neurobiology of perception and consciousness has made a serious advance, including discoveries at the (sub)cellular level related to both content processing mechanisms and state control mechanisms (Aru et al., 2019). The need for an update of the theory became apparent. However, as is often the case, during the updating process the theory itself became a new one and with the help from colleagues essentially a collective one. Critically important for this advancement were experimental results from Matthew Larkum’s research team (Larkum, 2013; Larkum, Zhu, & Sakmann, 1999; Suzuki & Larkum, 2020; Takahashi, Oertner, Hegemann, & Larkum, 2016).
In what follows we elaborate on our theory of consciousness – the Dendritic Integration Theory (DIT) (Aru et al., 2020b) and show how it reconciles the traditional separation between content vs. state of consciousness and cortex-based vs. thalamocortical foundations of consciousness. This theory takes the view that understanding the pyramidal neurons of the cortex and their dendritic trees is central to explaining the flow and control of information relating to conscious processing (Aru et al., 2020b). In this endeavour, as the matter to be explained is how conscious experience emerges from the brain, our theory starts by describing the attributes and characteristics of conscious experience in order to find neural mechanisms capable of explaining how these subjective characteristics can be achieved by certain neurobiological mechanisms. We assume that the basic evolutionary blueprint of consciousness neurobiology is similar between humans and other mammalian species and thus the NCC of humans can be studied with a little help from the other animals possessing similar brain structures. Indeed, here we aim to show that recent studies done with mice have brought new life into thalamocortical theories of consciousness. In particular, the recent finding that certain thalamic nuclei affect how information is integrated within single cortical neurons (Suzuki & Larkum, 2020), highlights the tight relationship between thalamic modulation, cortical processing and consciousness. DIT was first presented in another recent publication (Aru et al., 2020b). Here, we focus on the relationship of DIT to thalamocortical theories of consciousness and in particular address the issue of states and contents of consciousness.
2 Desiderata: What do we seek to explain?
A theory of consciousness should explain properties of consciousness. There are no firmly established properties of consciousness that all agree upon. Hence, here we will first posit four phenomenological properties of consciousness that we see as important (see Tononi & Edelman, 1998; Ginsburg & Jablonka, 2019 for a similar approach; Koch, 2020; Tononi & Koch, 2015). We do not think that the list is exhaustive or the best one (similarly we do not think that any other list is better). It is simply a list that helps us think more clearly about consciousness and can steer the search for the mechanisms of consciousness.
(1) Consciousness is mentally rich and multifaceted. Conscious experience captures different mental experiences such as feelings, emotions, sensations, perceptions, memories, imagery, agency, etc; also, it embraces sensations, perceptions and imagery in all sensory modalities.
(2) Conscious experience is integrated (see also Tononi & Edelman, 1998; Tononi, Boly, Massimini, & Koch, 2016). For example, in vision, all individual features differentiating the objects included in the field of subjective experience belong to the same unified field. (However, see Bayne, 2010; Pinto, Haan, & Lamme, 2017 for why in some cases phenomenal unity may become disintegrated.)
(3) Consciousness is continuous. When awake, alert and healthy, consciousness presents itself in a seamless subjective flow. Conscious perception is not lost when the eyes blink and conscious experience is continuous despite the saccadic eye movements (Aru, 2019).
(4) State of consciousness is multidimensional. The state of consciousness can vary along many behavioural and cognitive dimensions, including the measurable subjective dimensions (e.g., arousal, motor reactions, richness and vividness of experienced contents, metacognitive confidence, agency) (Bayne, Hohwy, & Owen, 2016).
The next six characteristics have been observed empirically and add important constraints to any neurobiological theory of consciousness:
(5) There is an interaction between the state and contents of consciousness. The state of consciousness affects the contents of consciousness. The same feature or object of conscious perception can be experienced in different ways (e.g. with different vividness, completeness, stability, subjective duration etc.) in different states of consciousness (Bachmann, 2012; Bayne et al., 2016; Haque, Lobier, Palva, & Palva, 2020; Koivisto & Neuvonen, 2020).
(6) Mental information processing can also occur in a subliminal mode, meaning that not all sensory signals that are encoded and adequately responded to are necessarily consciously experienced. Only part of the contents represented in the brain becomes conscious. (For reviews see Kim & Blake, 2005; Kouider & Dehaene, 2007; Mitchell, 2006)
(7) Emergence of conscious experience of some content in response to a stimulus is delayed in time. There is a temporal latency between stimulation and its experience (for review see Bachmann, 2000).
(8) Conscious experience has a limited temporal resolution, meaning that if the presentation of different stimuli occurs too rapidly then some fail to be consciously experienced. This is evidenced in empirical phenomena like backward masking (for review Bachmann & Francis, 2013) and attentional blink (for review Martens & Wyble, 2010). Even when an identical stimulus is switched on and off intermittently at very high frequency, the multiplicity of stimulus replications will not be consciously perceived and the stimuli will fuse into a continuous experience (Watson, 1986).
(9) Conscious experience is modulated by attention. Attending to an image enhances its subjective contrast (Carrasco, Ling, & Read, 2004); attending to an afterimage makes it disappear faster from conscious experience (Bachmann & Murd, 2010; Murd & Bachmann, 2011).
(10) Conscious experience of content depends on prior knowledge and expectations, it is context-dependent. The powerful effects of context-dependence are revealed in the fact that non-veridical representations of objects and events such as illusions or hallucinations are experienced even in the healthy brain (Aru & Bachmann, 2017; Aru, Tulver, & Bachmann, 2018; Kuhn & Rensink, 2016; Mack, Erol, Clarke, & Bert, 2016; Powers, Mathys, & Corlett, 2017).
This list can be extended to incorporate more features of and more results related to consciousness. However, we contend that these are the main characteristics a good scientific theory of consciousness should be able to accommodate. Can the DIT do this? Before assessing this question, we will explore the rich history of studying the influence of certain thalamic nuclei on consciousness.
3 A historical view on the role of thalamus and cortex in consciousness
3.1 Two types of thalamic projections to the cortex
We will not delve into the neuroanatomy of the thalamus (see Jones, 1985; Sherman & Guillery, 2001 for comprehensive overviews), but rather jump directly into the question how the thalamus is involved in the neurobiological processes underlying consciousness. For that we solely need to understand the difference between two types of thalamic projections to the cortex (Fig. 1).
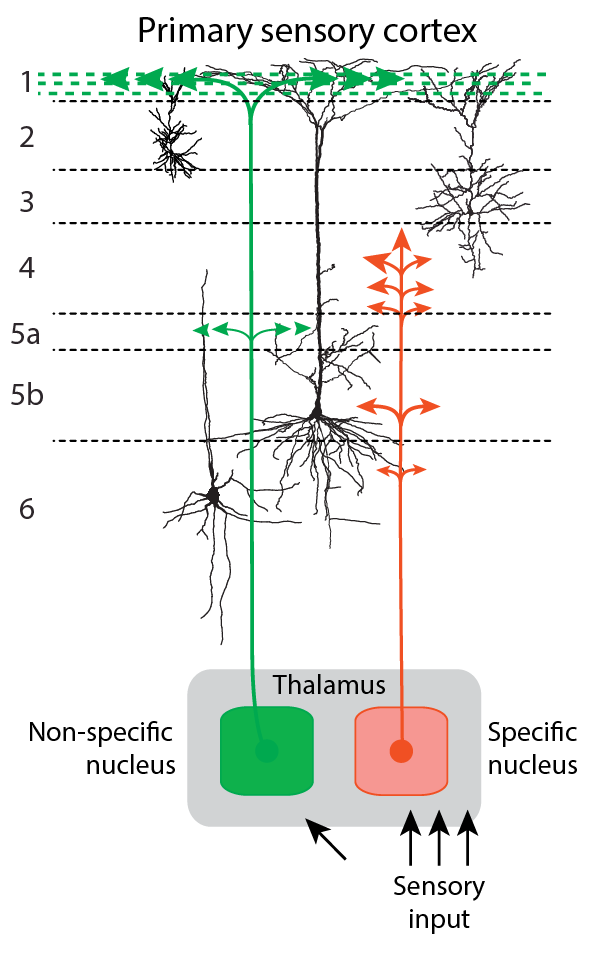
The thalamus is by no means only the relay of sensory information to the cortex. It also has other functions and this view is not new. The standard account of the thalamus held in the mid-20th century was that thalamus is a part of the reticulo-thalamic system with two general types of thalamocortical pathways (see Fig. 1): (1) the so-called specific pathways (SP) that carry afferent information to the cortex (e.g., via the lateral geniculate nucleus (LGN) in the case of vision) and (2) the so-called non-specific pathways (NSP) with large receptive fields that do not relay sensory content signals from receptors to cortex, but modulate the state of the cortical SP-neurons through diffuse cortical projections (Brazier, 1960; Brooks & Jung, 1973; Hassler, 1978; Kimble, 1977; Libet, 1993; Magoun, 1958; Mesulam, 2000; Moruzzi & Magoun, 1949; Newman, 1995).
The NSP-thalamus is an evolutionary supplement to the midbrain reticular formation and its role has been seen as the modulator of the state of cortical neural circuits, which allows transition between non-conscious states and alert states of consciousness (Brazier, 1960; Brooks & Jung, 1973; Magoun, 1958; Mesulam, 2000; Moruzzi & Magoun, 1949; Newman, 1995).
The NSP system is universal in that its modulatory effect extends to all sensory modalities and all classes of SP neurons. Importantly, receptive fields of SP and NSP can overlap: ascending afferents of both systems, SP and NSP converge on the same set of cortical neurons. A stimulus that drives a response in the SP system also evokes the activity of NSP (Brazier, 1960; Brooks & Jung, 1973; Magoun, 1958; Mesulam, 2000; Moruzzi & Magoun, 1949; Newman, 1995).
In the later years of the 20th century the SP vs NSP distinction underwent important developments (e.g., Jones, 1998, 2001). In earlier years the distinction between SP- and NSP-thalamus was delineated more in terms of clear structural differences and macroscopic circuit system differences. For example, in the visual system SP was more or less stringently associated with the LGN and NSP associated with certain thalamic intralaminar nuclei (e.g., Bogen, 1995a, 1995b; LaBerge, 1997; Newman, 1995; Purpura & Schiff, 1997; Ward, 2011). Subsequently, this difference became mainly associated with cell types independently of the borders of thalamic nuclei.
It turns out that thalamic cells can be characterized by immunoreactivity to different proteins and that this characterization generally captures the functional differences between SP and NSP systems as described above. Matrix cells are characterized by immunoreactivity to the calcium-binding protein D28K calbindin and project widely to superficial layers of the cerebral cortex. Their receptive field properties are similar to the classic NSP system and they generally receive subcortical inputs that differ from those of the SP pathways. The other type of thalamic neurons, the core cells are found in certain nuclei only. They project to middle layers of the cortex and target specific cortical areas. Hence they functionally correspond to the SP system of the older literature. Core cells are characterized by immunoreactivity to a different calcium-binding protein, parvalbumin (Jones, 1998, 2001). However, even this division is a simplification: there exist multi-specific thalamocortical projection neurons that have the specific projection pattern (i.e., to layer 4) in one cortical target area, but a non-specific projection pattern (i.e., to layers 1 and 5/6) in another area (Clascá, Porrero, Galazo, Rubio-Garrido, & Evangelio, 2016).
3.2 Empirical results supporting the role of thalamus in consciousness
Based on the classic views of the dual function of thalamus, thalamocortical theories of consciousness were introduced (Bachmann, 1984, 1998; Bogen, 1995a, 1995b; LaBerge, 1997; Llinás, Ribary, Contreras, & Pedroarena, 1998; Purpura & Schiff, 1997; Tononi & Edelman, 1998; Ward, 2011). Empirical evidence in support of the thalamic bases of (or at least decisive involvement in) consciousness is rich and varied.
Whether the brain is asleep or awake depends on NSP. Intralaminar thalamocortical neurons as part of the NSP increase their firing rate about 10 s before EEG desynchronization in natural transitions from slow-wave sleep to waking or REM sleep (Steriade, 1981). Thalamic deactivation is observed at sleep onset (Magnin et al., 2010). Thalamocortical sources send their excitatory signals up to cortex immediately before the REM sleep episodes, which typically include dream mentation (Steriade, Sakai, & Jouvet, 1984). Neocortical slow oscillations, characteristic of nonconscious states, occur after thalamocortical deafferentation (Lemieux, Chen, Lonjers, Bazhenov, & Timofeev, 2014). Honjoh and colleagues (Honjoh et al., 2018) showed that stimulation of matrix cells of the non-specific ventromedial (VM) thalamic nucleus in mice awoke the animal from NREM sleep and anesthesia. This stimulation also caused EEG activation in the high frequency band.
There are many known general anesthetics with different neural effects. However, virtually all of them have a common target in the thalamus (Alkire, Haier, & Fallon, 2000; Alkire, Hudetz, & Tononi, 2008). The fact that under general anesthesia primary sensory pathways remain functional and still communicate specific signals to cortex is adding credibility to the differentiation between SP- and NSP-functions. Electrical stimulation of NSP of the anesthetized experimental animals leads to desynchronization of the EEG with activity-patterns typical of the awake brain (Brazier, 1960; Moruzzi & Magoun, 1949; Munk, Roelfsema, König, Engel, & Singer, 1996).
Lesions localized in NSP typically cause absence of consciousness or distortion of conscious experiences in patients despite the fact that the SP system has remained intact (e.g., Kinney, Korein, Panigrahy, Dikkes, & Goode, 1994; Bogen, 1995a; Newman, 1995). Similarly, damage to NSP nuclei like the pulvinar leads to spatial neglect in humans (Karnath, Himmelbach, & Rorden, 2002; Ward, Danziger, Owen, & Rafal, 2002). Ischemia in the area supplied by the thalamo-subthalamic paramedian artery when extending to the paramedian thalamus (ventral posterior nuclei, dorsomedial, centromedian and central medial nuclei) shows itself clinically as impaired consciousness, hypersomnia, aphasia, amnesia and hemineglect (Seifert, Enzinger, Ropele, Storch, & Fazekas, 2004).
These three above-described classes of empirical facts show that the thalamus is in a position to hold the prime role in modulating the state and level of consciousness. Further research shows that the thalamus can also control the contents of consciousness. For example, it is possible to generate conscious contents by stimulating NSP without the normal sensory stimulation from the receptive field of the SP-units, which manifests in artificial sensations, phosphenes, and sometimes hallucinations (e.g., Gellhorn, 1961; Tasker, Organ, & Hawrylyshyn, 1980). The vividness of these experiences depends directly on the nature of NSP-stimulation. Furthermore, timing and subjective vividness of sensation and perception can be improved by NSP functional facilitation by psychopharmacological treatment with alert subjects (Brazier, 1960; Doty, Wilson, Bartlett, & Pecci-Saavedra, 1973).
The temporal latency of the modulatory NSP-system is longer than that of SP. Typical input delays in reaching cortical representational neurons are about 40–90 ms slower for NSP as compared to SP (Brazier, 1960; Brooks & Jung, 1973; Newman, 1995; Steriade, Jones, & Llinas, 1990). This is in line with the general knowledge about the delay of perceptual conscious experience in response to sensory stimuli (Bachmann, 2000).
SP-afferents show mainly non-branching axonal projections while axons from NSP-thalamus to cortex are richly branching, thus projecting also to the neighbouring SP-neurons (Bogen, 1995a; Newman, 1995; Purpura & Schiff, 1997; Steriade et al., 1990). This property puts the NSP modulation in a position to recruit context to support or contrast the SP content.
Lastly, the content of conscious experience is not only geared at the current environment but may also be retrieved from long-term or short-term memory. It is important to acknowledge that NSP-thalamus has a strong impact on memory formation and retrieval (Pereira de Vasconcelos & Cassel, 2015).
To sum up the previous sections, the research done in the last century already had demonstrated a clear relationship between the NSP-thalamus and consciousness. The thalamus was at the center of both experimental and theoretical interest in consciousness. However, despite the quite substantial data about the role of NSP-thalamus in consciousness, since the 1990s more emphasis has been put on the relationship between consciousness and cortical processing when looking for NCC. For example, although the neural global workspace theory (Dehaene & Changeux, 2011; Dehaene & Naccache, 2001; Mashour, Roelfsema, Changeux, & Dehaene, 2020) includes thalamocortical processing, it has a minor role compared to the cortical frontoparietal broadcasting system (Dehaene & Changeux, 2011; Mashour et al., 2020). Perhaps this change of perspective was caused by the fact that with microelectrode recordings and fMRI much evidence could be gathered about the role of cortical areas in consciousness (for review see Koch, Massimini, Boly, & Tononi, 2016; Mashour et al., 2020). Also, since the 1990s focus has been on studying and manipulating the contents of consciousness and the respective NCCs. The thalamus was somewhat put aside and assumed to provide the “enabling condition” for the contents of consciousness (Koch, 2004). The effects in the thalamus were often seen as secondary to those in the cortex (Alkire et al., 2008; Mashour, 2014).
In the following we want to offer a view that reconciles the thalamocortical and corticocortical perspectives (Aru et al., 2020b, 2019). In a nutshell we claim that thalamocortical and corticocortical processes mechanistically interact at the level of cortical layer 5 pyramidal (L5p) cells where single L5p neurons receive input from both, thalamic and different cortical sources. Thalamocortical interaction at the level of L5p cells is not limited to a simple “enabling function”, but forms the basis of igniting contents into consciousness as well as modulating the level of experience of these contents.
4 Thalamocortical interactions: an update
4.1 Cortical pyramidal cells couple cortical and thalamocortical interactions
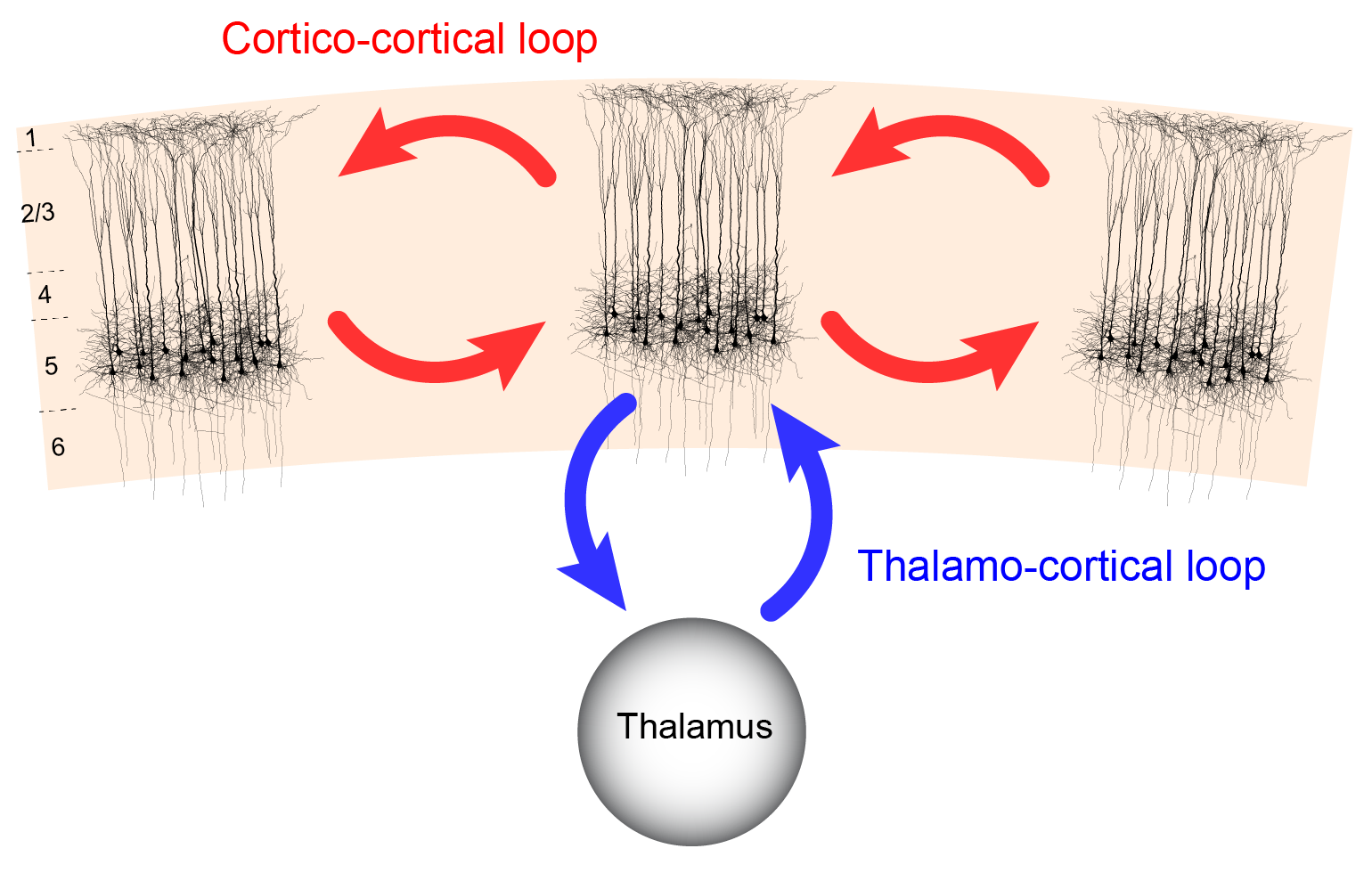
Crucial information for understanding the relationship between the thalamocortical system and consciousness has been obtained from the studies of cortical pyramidal cells (Aru et al., 2020b, 2019). L5p cells are attractive counterparts for any theory of cortical functioning because of their ability to integrate information across the cortical sheet. Their cell bodies lie in layer 5, but their dendrites span through all cortical layers. The apical dendrites of pyramidal cells mainly receive input from layer 1. The basal dendrites nearer to the soma mainly collect their inputs from layers 4-6. The layer 5B thick tufted pyramidal cells are the main output of the cortex, sending axons to thalamus, striatum and brainstem (Harris & Shepherd, 2015). While other neurons (e.g. certain types of layer 6 neurons) also project to the thalamus, layer 5B cells are thought to be the only types of cortical cells that drive thalamic activity (Sherman & Guillery, 2001). Hence, it has long been suspected that these cells also have a central role in consciousness (Ramon y Cajal, 1894).
Cortical L5p neurons have two functionally distinct sites of integration, one in the soma (somatic compartment) and one near the top of their apical trunk (apical compartment) (Aru et al., 2020b; Larkum, 2013). These compartments are known to be both functionally and anatomically quite distinct (Aru et al., 2020b; Larkum, 2013). The apical tuft receives diverse input from higher cortical areas and non-specific thalamic nuclei whereas the basal dendrites receive feedforward information from lower level cortical areas and first-order thalamic nuclei (Aru et al., 2020b; Larkum, 2013). It has been proposed that this segregation of two anatomical types of inputs correspond to the segregation of two functional types of inputs: context and sensory data (Aru et al., 2020b; Larkum, 2013; Phillips, 2017). L5p cells contain specific biophysical mechanisms that amplify the match between these two data streams (Larkum, 2013; Larkum et al., 1999). Hence, the L5p cells are pivotal in linking feedforward and feedback signalling pathways that are necessary for contextually modulated perception (Larkum et al., 1999; Takahashi et al., 2016; Xu et al., 2012). This notion is especially significant bearing in mind the growing theoretical consensus that contents of conscious perception are heavily determined by contextual information and not only by sensory afference (see characteristic 10 in our list of consciousness characteristics). This general view is consistent with some recent research conducted at a single-cell level.
Using a sensory detection task, Takahashi et al. (2016) showed that manipulation of apical dendritic activity of L5p cells had an effect on the behavioral report of the animal. Mice learned to detect weak whisker stimuli of different near-threshold magnitudes. Psychometric curves for whisker stimulation detection were delineated and correlated with neurometric curves. The activity of the apical tuft dendrites was monitored by performing fast-scanning two-photon Ca2+ imaging. The Ca2+ signal of apical dendrites was found to correlate with the behavior of the animal. Additionally, the apical dendritic signals predicted the behavioral hits and misses of threshold stimuli. Most importantly, direct modulation of the dendritic activity through pharmacological intervention or optogenetics had a measurable influence on the detection behavior of the animal, evidenced by a shift of the psychometric curve. Optogenetic enhancement of apical dendritic activity also caused false alarms -- activating the apical compartment of L5p caused the animal to lick as if a whisker stimulus had been present (Takahashi et al., 2016). We interpret this as a demonstration of illusory conscious perception: the animal experienced an illusory stimulus that was not actually presented, just like humans have been shown to do when expecting a stimulus due to some context (Aru & Bachmann, 2017; Aru et al., 2018).
In other related experiments it was found that the positive relationship between dendritic activity and animal behavior is largely constrained to the pyramidal neurons in layer 5B (Takahashi et al., 2020). For our present purposes this fact is important because these cells in layer 5B are known to project to thalamic nuclei (Harris & Shepherd, 2015). While the layer 5A cells possess denser corticocortical projections, they were not so tightly correlated with the perceptual report of the animal (Takahashi et al., 2020). Hence, these findings add credibility to the idea that when it comes to consciousness, thalamocortical interactions matter more than corticocortical ones.
The studies described in the preceding parts suggest that L5p neurons have an important role in conscious perception of the alert animal. Remarkably, the Larkum team has also demonstrated a direct relationship between L5p cell activity and the state of consciousness. For example, Murayama & Larkum (2009) performed fiberoptic Ca2+ imaging of the apical dendrites in L5p cells to compare the effect of different states of consciousness on the activity of the apical compartment. Brief air-puffs were delivered to the hindlimb of the animal. The apical dendritic response to this stimulation was 4-fold stronger in the quiet awake state than in the anesthetized state. Thus, the awake state has a strong impact on the activity of L5p apical dendrites (Phillips et al., 2018). When the animal moved its hindlimbs in response to the air-puff - an indication of perceiving the air-puff -, the apical dendritic response was 14-fold stronger than under anesthesia, this activity stemming from a prolonged response duration. In other words, the change in the state of consciousness was associated with some (4-fold) increase in the activity of apical dendrites, whereas combining the state of consciousness with a particular content (air-puff) led to a massive (14-fold) increase of this activity (Murayama & Larkum, 2009). Hence, the massive increase in the duration of L5p apical dendritic activity reflects the interaction between the state and the contents of consciousness.
Evidence from research reviewed thus far shows that L5p cells are situated at the strategically central position to integrate corticocortical and thalamocortical processing (Aru et al., 2020b, 2019). These cells have two functionally distinct compartments. The apical compartment is integrating contextual information and modulations from diverse sources while the somatic compartment is mainly collecting feedforward information. Controlling the interaction between these two compartments within a pool of single cortical pyramidal cells could provide one key mechanism for thalamocortical interactions in linking contents and states of consciousness (Aru et al., 2020b, 2019).
4.2 Non-specific/higher-order thalamus modulates integration within single pyramidal cells
Recently, Suzuki & Larkum (2020) reported a relatively simple and elegant mechanism about how non-specific thalamic nuclei could gate the interaction between apical and somatic compartments. They were studying a long-standing mystery about consciousness and anesthetic-induced unconsciousness. Namely, how is it possible that consciousness can be disrupted while not substantially affecting the firing properties of cortical neurons in general and not switching off primary feedforward input to cortical areas?
The authors optogenetically stimulated the apical compartment of L5p cells and measured the effects of this perturbation on the electrophysiological activity at soma of the same cells, while varying the conscious state of the animal. In the awake state dendritic stimulation had a large effect on the soma and this manipulation led to high frequency firing of the neurons. However, various anesthetics made this effect disappear: the same optogenetic stimulation of the apical compartment did not propagate to the soma. Under anesthesia the soma was decoupled from any potential apical influences generated by top-down signals specifying the context. These results could explain why anesthesia leads to loss of consciousness (Fig. 3).
Suzuki & Larkum (2020) went on to study the processes underlying this effect: Which mechanisms are responsible for this decoupling between the apical and somatic compartments? It turned out that this coupling is gated by the non-specific thalamic nucleus, in this case the posteromedial nucleus (POm). The authors show that blocking metabotropic receptor activation arising from non-specific thalamic input decouples apical and somatic compartments in awake animals. In other words, anesthesia and down-regulation of metabotropic receptor activity along the apical dendrite have the same specific effect. Also, inactivation of the POm with muscimol (GABAA receptor agonist) led to a breakdown of this apical-to-soma signal propagation in awake animals, strongly suggesting that non-specific thalamic nuclei can control the interaction between the two compartments of cortical L5p neurons. This is important, as it gives a mechanistic role for the thalamus in controlling cortical processing. In our view, this result demonstrates that corticocortical and thalamocortical theories of consciousness each contain a part of the story and that these two types of interactions are brought together at the level of cortical pyramidal neurons.
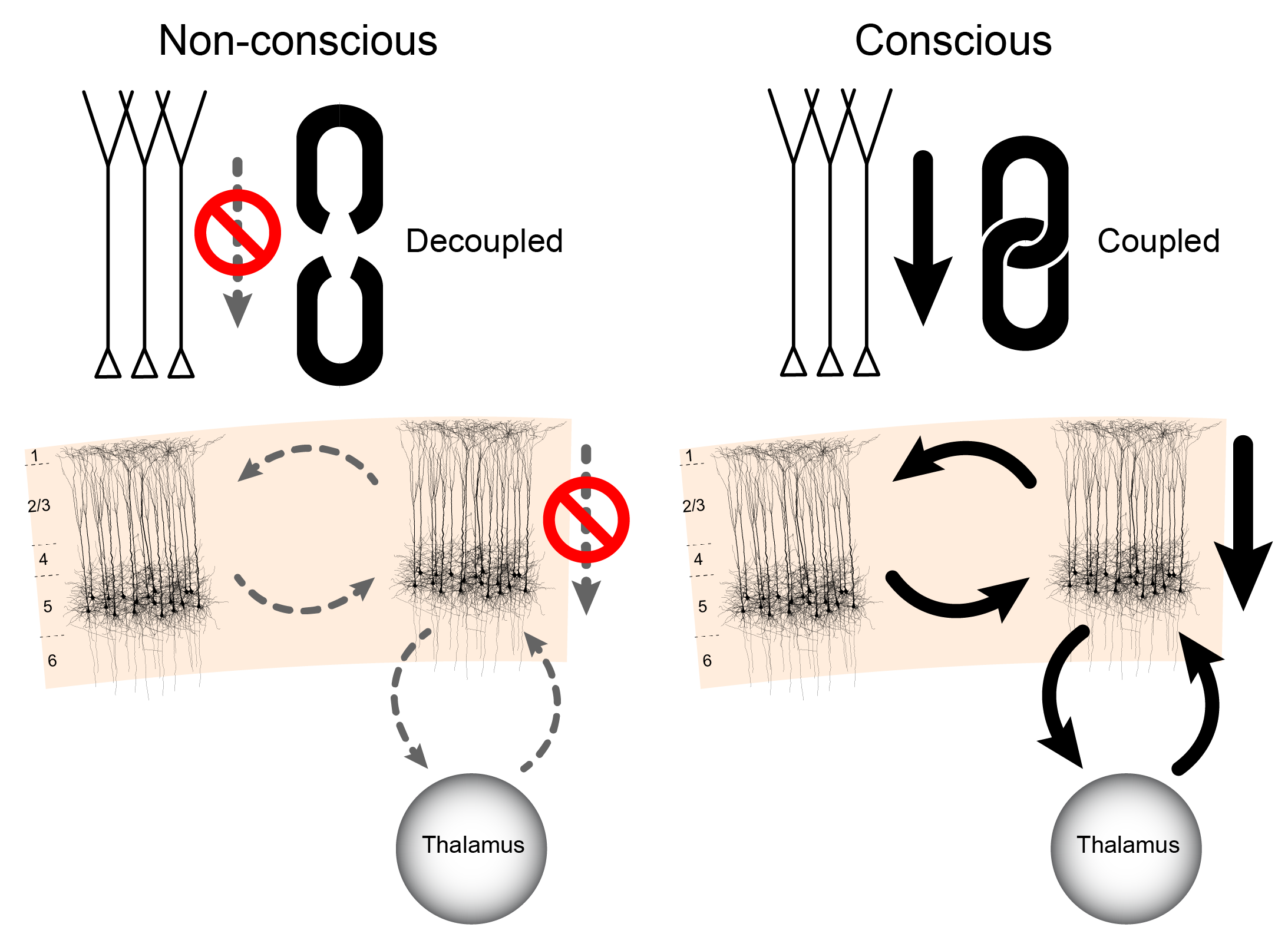
5 State and content of consciousness: the Dendritic Integration Theory
The key idea we want to stress in this paper is that the mechanisms of content and state of consciousness are basically overlapping and interaction between the state and the content of consciousness happens on the level of single pyramidal neurons (Aru et al., 2019). This perspective offers a natural way of understanding how state and content of consciousness interact in the brain. Moreover, it suggests that when studying consciousness it is not possible to write off the thalamus simply as an “enabling” factor: the thalamus controls the content represented in the pyramidal neurons by allowing it to “enter” consciousness. L5p cells as the units interacting both with thalamus and other cortical areas are critical for consciousness because of this dual role. We claim that the intertwinement of state and content of consciousness arises at the level of single L5p neurons because they hold a central position in both thalamocortical and corticocortical loops. These principles lay the backbone of the dendritic integration theory (DIT) (Aru et al., 2020b).
The direct relationship between L5p cell activity and the state of consciousness has been observed in Murayama & Larkum (2009), described earlier. With regard to the relation of L5p to the contents of perception, Takahashi et al. (2016) found that upregulation of apical dendritic activity of L5p cells enhanced the detection performance of the animal. Hudetz, Pillay, Wang, & Lee (2020) showed by intracortical in-vivo recordings that anesthesia disrupts cortical layer-specific top-down interactions between layer 3 and layer 5 of content-carrying neurons of the visual cortex. Marshel et al. (2019) demonstrated that stimulation of rodent V1 orientation-selective neurons caused asymmetric propagation of activity from layer 2/3 to layer 5, with layer 5 stimulation being more effective in facilitating orientation-discrimination behavior. To put these sets of evidence together, we can say that both corticocortical and thalamocortical theories of consciousness are correct to some extent, but the full characterization of the state and content of consciousness requires the understanding of how these two loops are coupled. Supported by the results of Suzuki & Larkum (2020) we have now more confidence in proposing here that the coupling between the corticocortical and thalamocortical loops happens at the level of single cortical L5p neurons.
To recap, these neurons, especially the thick-tufted layer 5B neurons have the following crucial properties. First, the thick-tufted layer 5B neurons are the key cortical output neurons projecting also to non-specific thalamic nuclei (Harris & Shepherd, 2015; Sherman & Guillery, 2001). This means that the results of the cortical computations will be propagated from cortex to the non-specific thalamic nuclei through layer 5B neurons. As the non-specific thalamic nuclei have diffuse projection patterns across the whole cortex, a strong signal from a few cortical columns could reach the whole thalamocortical system through the thalamic “hub”.
Second, the apical compartment of thick-tufted layer 5B neurons is a convergence zone for corticocortical and thalamocortical information (Aru et al., 2020b; Larkum, 2013). As the apical processing can have a dramatic effect on the spiking output of these neurons via dendritic Ca2+ spikes (Larkum, 2013; Larkum et al., 1999), anything that is communicated by the layer 5B neurons is contextually modulated (Phillips, 2017). Also, completing the loop, these layer 5B neurons receive projections from the same non-specific thalamic nuclei they project to. These projections target layers 1 and 5A (Larkum, 2013).
Finally, the research by Suzuki & Larkum (2020) demonstrates that the input to the apical dendrites of L5p neurons does not propagate to the soma of these cells under anesthesia. Hence, under anesthesia the contextually modulated information provided to L5p apical compartment does not reach non-specific thalamic nuclei and hence is also not communicated to the rest of the thalamocortical system. Importantly for the theme of the present paper, when Suzuki & Larkum (2020) artificially suppressed a higher order thalamic nucleus (POm) in the awake state, propagation of activity from the apical compartment to the somatic compartment was also disrupted, similarly to what was observed in the anesthetic state. These observations support the view that consciousness depends on both corticocortical and thalamocortical connectivity.
According to DIT the corticocortical loop is indeed essential for the computation of the contents of consciousness. Yet, without being integrated to the thalamocortical system, this corticocortical processing is unconscious. The thalamocortical loop is necessary to integrate the contextually modulated cortical processing into conscious experience. We claim here that conscious perception of the environment or internal thought requires the loop between the apical and the somatic compartments of L5p neurons and the non-specific thalamus, which projects back to the apical dendrites of the same L5p neurons.
DIT suggests that consciousness is associated with the integration of information streams impinging on the apical and basal compartments of L5p neurons (Aru et al., 2020b). As the apical stream carries context, the result of this integration is contextually modulated experience. Such dendritic integration couples thalamocortical and corticocortical loops (see Fig. 3) and propagates this contextually modulated experience to the whole thalamocortical system (Aru et al., 2020b). The mechanism discovered by Suzuki & Larkum (2020) could be the crucial switch for controlling the propagation within this loop (Fig. 3).
5.1 DIT and the phenomenological properties of consciousness
Next we will see how DIT is compatible with the key phenomenological characteristics of consciousness as listed above. Note that we are not claiming that no other theory can explain these properties. We simply demonstrate how DIT naturally accounts for them.
(1) Consciousness is mentally rich and multifaceted. Given that specific content is mainly processed in specialized cortical areas (for review Koch et al., 2016; Mashour et al., 2020), it is important to acknowledge that thalamic efferents, especially those from the NSP-thalamus, innervate all areas of the cortex (Jones, 1985; Sherman & Guillery, 2001) and are thus in a position to modulate such content-specific processing.
(2) Conscious experience is integrated. The thalamus is in the central position to integrate the various aspects of processing happening at different cortical nodes. It is densely connected to all areas of the cortex between and within modally specified regions (Jones, 1985; Sherman & Guillery, 2001).
(3) Consciousness, when fully present, is continuous. Even though specific contents can change hand-in-hand with the specific L5p neurons that are currently active, consciousness is continuous because at any given moment there are some reverberations in the thalamocortical system (Aru et al., 2020b). In other words, while the combination of L5p neurons participating in conscious experience changes, the integration happening with these neurons is always supported by the NSP-thalamus; the same mechanism works also for the unchanging content. In short, continuity of consciousness comes from the continuity of the non-specific mechanism that modulates specific contents.
(4) State of consciousness is multidimensional. As explained above, DIT proposes that consciousness arises from the interactions of one data stream impinging on the apical compartment, another stream affecting the basal compartment, and the NSP-thalamus that controls the interaction between these streams. These three ingredients (apical compartment, basal compartment and the NSP-thalamus) could be seen as three dimensions of the state of consciousness. For example, it has been proposed that during dreaming the apical compartment can generate conscious experience with a minor contribution from the basal compartment (Aru et al., 2020a). Perhaps the dimension of arousal is controlled by the NSP-thalamic input to the L5p neurons.
5.2 DIT and the empirical characteristics of consciousness
In the introduction we also proposed six empirical characteristics that any theory of consciousness should explain.
(5) There is an interaction between the state and contents of consciousness: A theory of consciousness should explain how the state of consciousness affects the contents of consciousness. DIT offers a natural explanation: Contents are processed by the respective L5p neurons in cortex; NSP-thalamus, a structure traditionally associated with the state of consciousness, modulates the integration of the different compartments of the L5p neurons (Aru et al., 2020b; Suzuki & Larkum, 2020). Thus, the state of consciousness affects the contents of consciousness and this interaction happens along the apical dendrites of L5p neurons (Aru et al., 2019).
(6) Processing of mental information can proceed also in its subliminal mode. According to the theory presented here, if the activity in cortical areas is insufficiently propagated to the non-specific thalamic nuclei, this processing will not be conscious. In other words, we make a strong prediction that cortical processing in itself, when not integrated with the non-specific neurons of thalamus, is not conscious. In particular, feedforward cortical processing, where information is mainly flowing on the superficial layers bypassing layer 5 neurons, will be non-conscious. Such non-conscious processing can still contribute to our behavior and responses (For reviews see Kim & Blake, 2005; Kouider & Dehaene, 2007; Mitchell, 2006). This thalamocortical perspective could also shed new light on some debates about which cortical areas are crucial for consciousness. We claim that there are no specific cortical areas that are crucial for consciousness, it is the type of interaction with non-specific thalamic nuclei that matters (Aru et al., 2020b; Suzuki & Larkum, 2020). Some areas like the prefrontal cortex and the parietal association areas have particularly strong connectivity to the non-specific thalamic nuclei (Halassa & Kastner, 2017; Jones, 1985) and hence their activity difference is expected to be more found when the contrast between trials with and without conscious perception is applied (Aru, Bachmann, Singer, & Melloni, 2012). In short, the theory claims that cortical processing without the propagation to non-specific thalamic nuclei will be non-conscious.
(7) There is a temporal latency between the initial cortical representation of a content and the corresponding conscious experience. Stimuli need some time to be incorporated into the thalamocortical loop. According to some more classic thalamocortical theories of consciousness, this latency comes from the fact that the non-specific nuclei do not receive direct sensory input and hence respond only after the respective cortical areas have been activated by feedforward routes (Bachmann, 2000). According to the present view conscious perception of a content requires the loop between the apical dendrites of L5p neurons, the soma of these cells and the non-specific thalamus, which projects back to the apical dendrites. Igniting this loop takes time and therefore there is a latency from the onset of the cortical processing of a particular content to the conscious experience of that content. Recent NCC research capitalizing on contrastive analysis sets the lower limit of this latency at about 100-200 ms (Förster, Koivisto, & Revonsuo, 2020).
(8) Conscious experience has a limited temporal resolution. This characteristic is tightly related to the previous one: if any conscious perception is based on the loop we have described, then it is hard for conscious perception to resolve anything that happens faster than the processing time of this loop. In other words we claim that the temporal resolution of conscious experience stems from the propagation time within the thalamocortical loop. An explanation for experimental phenomena like backward masking has also been provided by more classic and more recent thalamocortical theories of consciousness. The novel findings gained over the last decades can help us better understand the specifics of these mechanisms. For example, in masking two successive stimuli are presented at the same spatial location and, under certain conditions, the second stimulus (“the mask”) can completely abolish the first (“the target”) from consciousness (Bachmann, 2000). So, although the target is presented 50-70 ms before the mask, it is not consciously perceived. According to the present theory, this effect is obtained as follows: the first stimulus activates L5p neurons and hence starts the loop, but by the time the activity propagates from the L5p neurons to the non-specific thalamus and back to the apical dendrites of L5p neurons, the second stimulus has arrived in cortex and now “steals the fire” ignited by the first stimulus. The first stimulus starts the loop, but the following stimulus benefits from it.
(9) Conscious experience is modulated by attention. Attention has been linked to neurobiological processes happening in thalamus for quite some time (Crick, 1984). There are several ways to understand attention in the present framework. First, attention could be controlling the firing activity of cortical pyramidal cells through the modulation of activity at the apical compartment of the selective set of content carrying neurons. Second, the main signal for attentional selection could come from subcortical areas like the superior colliculus that project to thalamus (Krauzlis, Lovejoy, & Zénon, 2013; Wurtz, McAlonan, Cavanaugh, & Berman, 2011). Finally, it is possible that changes in attention may be associated with changes in the firing activity of L5p by regulating the coupling of two compartments via some non-specific thalamic nuclei (Suzuki & Larkum, 2020). Whichever mechanism turns out to be the best for capturing the effects of attention, the thalamus is in the position to affect cortical processing in the form of attentional effects. As we and many others have believed that research supports the argument that attention is best conceptualized as a process distinct from consciousness (Aru & Bachmann, 2013; Koch & Tsuchiya, 2007; Lamme, 2004), we would expect that the neural mechanisms of attention are also different from the loop of consciousness, despite the fact that these mechanisms easily interact.
(10) Conscious experience relies on prior knowledge and expectations, it is context-dependent. As explained above, contextual information mainly targets the apical compartment of cortical pyramidal cells. By gating the interaction between the apical and the somatic compartment the non-specific thalamus can control whether this prior knowledge is incorporated into conscious experience or not.
5.3 Questions for further research
While the mechanism demonstrated by Suzuki & Larkum (2020) is elegant, several questions remain. The key question is whether the demonstrated thalamocortical gating is a condition to regulate the general state of consciousness only or can it be used to selectively gate specific alternative contents – e.g., allow certain activity patterns that carry specific contents to enter or keep from entering consciousness. While it was shown that in the alert state thalamic activity allows for the direct propagation of apical activity into the soma, it is presently unclear whether during alertness this modulation is homogenous all over cortex (i.e., the apical compartments of all pyramidal neurons in the cortex are permitted to influence the somas) or whether this gating mechanism can act more locally (i.e. allow the apical activity to propagate on certain areas of the cortex only). If the latter is the case, work needs to demonstrate how local exactly this modulation can be – can it modulate coupling between the compartments of pyramidal neurons on the level of cortical columns? One earlier model of nonspecific thalamocortical modulation necessary for consciously experiencing the content of conscious perception assumed the overlapping of receptive fields for specific cortical content-neurons and non-specific subcortical “consciousness-providing” neurons (e.g., Bachmann, 1994). It is by no means forbidden to regard overlapping spatial locations of objects as a form of spatial-location context in addition to other forms of context such as categorical associations, priming based on conditioning, or personal relevance related attentional tuning.
Suzuki & Larkum (2020) showed that the (de)coupling mechanism is also sensitive to acetylcholine (ACh) blockers that significantly reduced the wakefulness-enhanced somatic responses of the cell whereas noradrenaline (NA) blockers did not (although NA still modulated dendritic responses). The effect of ACh on coupling is consistent with its previously found effects on neuronal properties (Goard & Dan, 2009; Polack, Friedman, & Golshani, 2013). However, the absence of an effect of NA blockers on the propagation from the apical dendrites to the soma may seem inconsistent with the wide set of data on strong involvement of NA based neuromodulation in supporting consciousness (Carter et al., 2010; Gelbard-Sagiv, Magidov, Sharon, Hendler, & Nir, 2018; Phillips, Bachmann, & Storm, 2018). Since the excitability of apical compartment of L5p neurons is enhanced by NA (Labarrera et al., 2018; Suzuki & Larkum, 2020), NA may contribute to the propagation of slower responses (involving Ca2+ spikes) to contextual inputs arriving at the apical compartment. For example, the evidence that NA modulates liminal perceptual sensitivity and accuracy (i.e., determining whether the content is consciously perceived or not), as well as late visually evoked EEG and fMRI responses, supports an important enabling role for noradrenergic modulation in perceptual awareness (Gelbard-Sagiv et al., 2018). It is generally known that EEG correlates of conscious perception are relatively slow, emerging after about 100–200 ms post stimulus (Bachmann, 1994; Förster et al., 2020; Rutiku & Bachmann, 2017). NA phasic activity timescale nicely corresponds to this perceptual timescale (Totah, Logothetis, & Eschenko, 2019) and often facilitates perception (Waterhouse & Navarra, 2019). Furthermore, NA axons in primary cortex of the awake mice show multimodal effects: phasic responses to stimulation in one modality were accompanied by phasic responses in other modalities (e.g., associating somatosensory and visual sensory effects) (Deitcher, Leibner, Kutzkel, Zylbermann, & London, 2019). Thus, NA neuromodulation seems to be multimodally contextual.
On the other hand, involvement of ACh in anesthesia vs wakeful perception regulation as well as in mediating fast conscious perception, cannot be overlooked either. For instance, recent work supports the view that cholinergic cortical response to stimulation can be phasic and with temporal resolution allowing reaction after 200 ms (Disney & Higley, 2020; Sarter & Lustig, 2020), which roughly corresponds to temporal resolution of the NA post-stimulus activity. The critical role of ACh as compared to NA was recently suggested by Pal et al. (2020): Prefrontal cholinergic stimulation of the anesthetized rat, but not noradrenergic stimulation, caused awake-like reactions. EEG activation was similar for both of these interventions, however, the righting reflex, a behavioral measure of unconsciousness in rats, and some mobility was restored only by ACh. While these authors relate consciousness to ACh modulation and not NA modulation, there is a clear possibility that behavioral reactions are performed in a zombie mode without the concomitant perceptual experience and EEG changes reflected regaining some sensitivity, but in a state akin to what in humans is called the locked-in state. Future research must add more understanding of the exact relative roles of ACh and NA in the control of conscious states and contents.
6 Conclusion
In the present work we have attempted to update the classic thalamocortical theories of consciousness in the light of novel insights from circuits neuroscience. We tried to offer a perspective where the corticocortical and thalamocortical theories can be reconciled: these processes interact at the level of single cortical pyramidal cells. Non-specific thalamic nuclei affect the coupling between the apical and somatic compartments of the pyramidal cells, thereby coupling or decoupling the thalamocortical loop. By virtue of the present conceptualization, it becomes clear why consciousness can be manipulated in either way – by cortical and by thalamic interventions. It is clear from our analysis that the thalamocortical system possesses the characteristics of a system corresponding to conscious experience.
Acknowledgments
We are thankful to Matthew Larkum for comments on this manuscript. JA was supported by the European Union’s Horizon 2020 Research and Innovation Programme under the Marie Skłodowska-Curie Grant Agreement No. 799411. MS was supported by German Research Foundation EXC 257.
References
Alkire, M. T., Haier, R. J., & Fallon, J. H. (2000). Toward a unified theory of narcosis: Brain imaging evidence for a thalamocortical switch as the neurophysiologic basis of anesthetic-induced unconsciousness. Consciousness and Cognition, 9(3), 370–386. https://doi.org/10.1006/ccog.1999.0423
Alkire, M. T., Hudetz, A. G., & Tononi, G. (2008). Consciousness and anesthesia. Science, 322(5903), 876–880. https://doi.org/10.1126/science.1149213
Aru, J. (2019). From aliens to invisible limbs: The transitions that never make it into conscious experience. In G. Hesselmann (Ed.), Transitions between consciousness and unconsciousness (pp. 148–162). Routledge.
Aru, J., & Bachmann, T. (2013). Phenomenal awareness can emerge without attention. Frontiers in Human Neuroscience, 7. https://doi.org/10.3389/fnhum.2013.00891
Aru, J., & Bachmann, T. (2017). Expectation creates something out of nothing: The role of attention in iconic memory reconsidered. Consciousness and Cognition, 53, 203–210. https://doi.org/10.1016/j.concog.2017.06.017
Aru, J., Bachmann, T., Singer, W., & Melloni, L. (2012). Distilling the neural correlates of consciousness. Neuroscience and Biobehavioral Reviews, 36(2), 737–746. https://doi.org/10.1016/j.neubiorev.2011.12.003
Aru, J., Siclari, F., Phillips, W. A., & Storm, J. F. (2020a). Apical drive—A cellular mechanism of dreaming? Neuroscience & Biobehavioral Reviews. https://doi.org/10.1016/j.neubiorev.2020.09.018
Aru, J., Suzuki, M., & Larkum, M. E. (2020b). Cellular mechanisms of conscious processing. Trends in Cognitive Sciences, 24(10), 814–825. https://doi.org/10.1016/j.tics.2020.07.006
Aru, J., Suzuki, M., Rutiku, R., Larkum, M. E., & Bachmann, T. (2019). Coupling the state and contents of consciousness. Frontiers in Systems Neuroscience, 13. https://doi.org/10.3389/fnsys.2019.00043
Aru, J., Tulver, K., & Bachmann, T. (2018). It’s all in your head: Expectations create illusory perception in a dual-task setup. Consciousness and Cognition, 65, 197–208. https://doi.org/10.1016/j.concog.2018.09.001
Bachmann, T. (1984). The process of perceptual retouch: Nonspecific afferent activation dynamics in explaining visual masking. Perception & Psychophysics, 35(1), 69–84. https://doi.org/10.3758/bf03205926
Bachmann, T. (1994). Psychophysiology of visual masking: The fine structure of conscious experience. Nova Science Publishers.
Bachmann, T. (1998). Fast dynamics of visibility of brief images: The perceptual-retouch viewpoint. In S. R. Hameroff, A. W. Kaszniak, & A. C. Scott (Eds.), Toward a science of consciousness ii. The second Tucson discussions and debates. (pp. 345–359). The MIT Press.
Bachmann, T. (2000). Microgenetic approach to the conscious mind. John Benjamins Publishing Company.
Bachmann, T. (2012). How to begin to overcome the ambiguity present in differentiation between contents and levels of consciousness? Frontiers in Psychology, 3. https://doi.org/10.3389/fpsyg.2012.00082
Bachmann, T., & Francis, G. (2013). Visual masking: Studying perception, attention, and consciousness. Elsevier Science.
Bachmann, T., & Murd, C. (2010). Covert spatial attention in search for the location of a color-afterimage patch speeds up its decay from awareness: Introducing a method useful for the study of neural correlates of visual awareness. Vision Research, 50(11), 1048–1053. https://doi.org/10.1016/j.visres.2010.03.013
Bayne, T. (2010). The unity of consciousness. Oxford University Press.
Bayne, T., Hohwy, J., & Owen, A. M. (2016). Are there levels of consciousness? Trends in Cognitive Sciences, 20(6), 405–413. https://doi.org/10.1016/j.tics.2016.03.009
Bogen, J. E. (1995a). On the neurophysiology of consciousness: I. An overview. Consciousness and Cognition, 4(1), 52–62. https://doi.org/10.1006/ccog.1995.1003
Bogen, J. E. (1995b). On the neurophysiology of consciousness: Part II. constraining the semantic problem. Consciousness and Cognition, 4(2), 137–158. https://doi.org/10.1006/ccog.1995.1020
Brazier, M. A. B. (1960). Electrical activity of the nervous system. Baltimore.
Brooks, B., & Jung, R. (1973). Neuronal physiology of the visual cortex. In G. Berlucchi, G. S. Brindley, B. Brooks, O. D. Creutzfeldt, E. Dodt, R. W. Doty, et al. (Eds.), Visual centers in the brain (pp. 325–440). https://doi.org/10.1007/978-3-642-65495-4_9
Carrasco, M., Ling, S., & Read, S. (2004). Attention alters appearance. Nature Neuroscience, 7(3), 308–313. https://doi.org/10.1038/nn1194
Carter, M. E., Yizhar, O., Chikahisa, S., Nguyen, H., Adamantidis, A., Nishino, S., et al. (2010). Tuning arousal with optogenetic modulation of locus coeruleus neurons. Nature Neuroscience, 13(12), 1526–1533. https://doi.org/10.1038/nn.2682
Clascá, F., Porrero, C., Galazo, M. J., Rubio-Garrido, P., & Evangelio, M. (2016). Chapter 4 - anatomy and development of multispecific thalamocortical axons: Implications for cortical dynamics and evolution. In K. S. Rockland (Ed.), Axons and brain architecture (pp. 69–92). https://doi.org/10.1016/B978-0-12-801393-9.00004-9
Crick, F. (1984). Function of the thalamic reticular complex: The searchlight hypothesis. Proceedings of the National Academy of Sciences, 81(14), 4586–4590. https://doi.org/10.1073/pnas.81.14.4586
Dehaene, S., & Changeux, J.-P. (2011). Experimental and theoretical approaches to conscious processing. Neuron, 70(2), 200–227. https://doi.org/10.1016/j.neuron.2011.03.018
Dehaene, S., & Naccache, L. (2001). Towards a cognitive neuroscience of consciousness: Basic evidence and a workspace framework. Cognition, 79(1), 1–37. https://doi.org/10.1016/S0010-0277(00)00123-2
Deitcher, Y., Leibner, Y., Kutzkel, S., Zylbermann, N., & London, M. (2019). Nonlinear relationship between multimodal adrenergic responses and local dendritic activity in primary sensory cortices. bioRxiv. https://doi.org/10.1101/814657
Disney, A. A., & Higley, M. J. (2020). Diverse spatiotemporal scales of cholinergic signaling in the neocortex. Journal of Neuroscience, 40(4), 720–725. https://doi.org/10.1523/JNEUROSCI.1306-19.2019
Doty, R. W., Wilson, P. D., Bartlett, J. R., & Pecci-Saavedra, J. (1973). Mesencephalic control of lateral geniculate nucleus in primates. I. Electrophysiology. Experimental Brain Research, 18(2), 189–203. https://doi.org/10.1007/BF00234723
Förster, J., Koivisto, M., & Revonsuo, A. (2020). ERP and MEG correlates of visual consciousness: The second decade. Consciousness and Cognition, 80, 102917. https://doi.org/10.1016/j.concog.2020.102917
Gelbard-Sagiv, H., Magidov, E., Sharon, H., Hendler, T., & Nir, Y. (2018). Noradrenaline modulates visual perception and late visually evoked activity. Current Biology, 28(14), 2239–2249.e6. https://doi.org/10.1016/j.cub.2018.05.051
Gellhorn, E. (1961). Cerebral interactions: Simultaneous activation of specific and unspecific systems. In Electrical stimulation of the brain (pp. 321–328). University of Texas Press.
Ginsburg, S., & Jablonka, E. (2019). The evolution of the sensitive soul: Learning and the origins of consciousness. MIT Press.
Goard, M., & Dan, Y. (2009). Basal forebrain activation enhances cortical coding of natural scenes. Nature Neuroscience, 12(11), 1444–1449. https://doi.org/10.1038/nn.2402
Halassa, M. M., & Kastner, S. (2017). Thalamic functions in distributed cognitive control. Nature Neuroscience, 20(12), 1669–1679. https://doi.org/10.1038/s41593-017-0020-1
Haque, H., Lobier, M., Palva, J. M., & Palva, S. (2020). Neuronal correlates of full and partial visual conscious perception. Consciousness and Cognition, 78, 102863. https://doi.org/10.1016/j.concog.2019.102863
Harris, K. D., & Shepherd, G. M. G. (2015). The neocortical circuit: Themes and variations. Nature Neuroscience, 18(2), 170–181. https://doi.org/10.1038/nn.3917
Hassler, R. (1978). Interaction of reticular activating system for vigilance and the truncothalamic and pallidal systems for directing awareness and attention under striatal control. In Cerebral correlates of conscious experience (pp. 111–129). North-Holland.
Honjoh, S., Sasai, S., Schiereck, S. S., Nagai, H., Tononi, G., & Cirelli, C. (2018). Regulation of cortical activity and arousal by the matrix cells of the ventromedial thalamic nucleus. Nature Communications, 9(1), 2100. https://doi.org/10.1038/s41467-018-04497-x
Hudetz, A. G., Pillay, S., Wang, S., & Lee, H. (2020). Desflurane anesthesia alters cortical layer-specific hierarchical interactions in rat cerebral cortex. Anesthesiology, 132(5), 1080–1090. https://doi.org/10.1097/ALN.0000000000003179
Jones, E. G. (1985). The thalamus. Springer US.
Jones, E. G. (1998). Viewpoint: The core and matrix of thalamic organization. Neuroscience, 85(2), 331–345. https://doi.org/10.1016/s0306-4522(97)00581-2
Jones, E. G. (2001). The thalamic matrix and thalamocortical synchrony. Trends in Neurosciences, 24(10), 595–601. https://doi.org/10.1016/s0166-2236(00)01922-6
Karnath, H. O., Himmelbach, M., & Rorden, C. (2002). The subcortical anatomy of human spatial neglect: Putamen, caudate nucleus and pulvinar. Brain: A Journal of Neurology, 125(2), 350–360. https://doi.org/10.1093/brain/awf032
Kim, C.-Y., & Blake, R. (2005). Psychophysical magic: Rendering the visible ’invisible’. Trends in Cognitive Sciences, 9(8), 381–388. https://doi.org/10.1016/j.tics.2005.06.012
Kimble, D. P. (1977). Psychology as a biological science. Scott Foresman & Co.
Kinney, H. C., Korein, J., Panigrahy, A., Dikkes, P., & Goode, R. (1994). Neuropathological findings in the brain of Karen Ann Quinlan. The role of the thalamus in the persistent vegetative state. The New England Journal of Medicine, 330(21), 1469–1475. https://doi.org/10.1056/NEJM199405263302101
Koch, C. (2004). The quest for consciousness: A neurobiological approach. Roberts & Company Publishers.
Koch, C. (2020). The feeling of life itself: Why consciousness is widespread but can’t be computed. MIT Press.
Koch, C., Massimini, M., Boly, M., & Tononi, G. (2016). Neural correlates of consciousness: Progress and problems. Nature Reviews. Neuroscience, 17(5), 307–321. https://doi.org/10.1038/nrn.2016.22
Koch, C., & Tsuchiya, N. (2007). Attention and consciousness: Two distinct brain processes. Trends in Cognitive Sciences, 11(1), 16–22. https://doi.org/10.1016/j.tics.2006.10.012
Koivisto, M., & Neuvonen, S. (2020). Masked blindsight in normal observers: Measuring subjective and objective responses to two features of each stimulus. Consciousness and Cognition, 81, 102929. https://doi.org/10.1016/j.concog.2020.102929
Kouider, S., & Dehaene, S. (2007). Levels of processing during non-conscious perception: A critical review of visual masking. Philosophical Transactions of the Royal Society of London. Series B, Biological Sciences, 362(1481), 857–875. https://doi.org/10.1098/rstb.2007.2093
Krauzlis, R. J., Lovejoy, L. P., & Zénon, A. (2013). Superior colliculus and visual spatial attention. Annual Review of Neuroscience, 36, 165–182. https://doi.org/10.1146/annurev-neuro-062012-170249
Kuhn, G., & Rensink, R. A. (2016). The vanishing ball illusion: A new perspective on the perception of dynamic events. Cognition, 148, 64–70. https://doi.org/10.1016/j.cognition.2015.12.003
Labarrera, C., Deitcher, Y., Dudai, A., Weiner, B., Kaduri Amichai, A., Zylbermann, N., & London, M. (2018). Adrenergic modulation regulates the dendritic excitability of layer 5 pyramidal neurons in vivo. Cell Reports, 23(4), 1034–1044. https://doi.org/10.1016/j.celrep.2018.03.103
LaBerge, D. (1997). Attention, awareness, and the triangular circuit. Consciousness and Cognition, 6(2-3), 149–181. https://doi.org/10.1006/ccog.1997.0305
Lamme, V. a. F. (2004). Separate neural definitions of visual consciousness and visual attention: A case for phenomenal awareness. Neural Networks: The Official Journal of the International Neural Network Society, 17(5-6), 861–872. https://doi.org/10.1016/j.neunet.2004.02.005
Larkum, M. (2013). A cellular mechanism for cortical associations: An organizing principle for the cerebral cortex. Trends in Neurosciences, 36(3), 141–151. https://doi.org/10.1016/j.tins.2012.11.006
Larkum, M. E., Zhu, J. J., & Sakmann, B. (1999). A new cellular mechanism for coupling inputs arriving at different cortical layers. Nature, 398, 338–341. https://doi.org/10.1038/18686
Lemieux, M., Chen, J.-Y., Lonjers, P., Bazhenov, M., & Timofeev, I. (2014). The impact of cortical deafferentation on the neocortical slow oscillation. The Journal of Neuroscience: The Official Journal of the Society for Neuroscience, 34(16), 5689–5703. https://doi.org/10.1523/JNEUROSCI.1156-13.2014
Libet, B. (1993). Neuronal vs. Subjective timing for a conscious sensory experience. In B. Libet (Ed.), Neurophysiology of consciousness (pp. 149–162). https://doi.org/10.1007/978-1-4612-0355-1_8
Llinás, R., Ribary, U., Contreras, D., & Pedroarena, C. (1998). The neuronal basis for consciousness. Philosophical Transactions of the Royal Society of London. Series B: Biological Sciences, 353(1377), 1841–1849. https://doi.org/10.1098/rstb.1998.0336
Mack, A., Erol, M., Clarke, J., & Bert, J. (2016). No iconic memory without attention. Consciousness and Cognition, 40, 1–8. https://doi.org/10.1016/j.concog.2015.12.006
Magnin, M., Rey, M., Bastuji, H., Guillemant, P., Mauguière, F., & Garcia-Larrea, L. (2010). Thalamic deactivation at sleep onset precedes that of the cerebral cortex in humans. Proceedings of the National Academy of Sciences of the United States of America, 107(8), 3829–3833. https://doi.org/10.1073/pnas.0909710107
Magoun, H. W. (1958). The waking brain. Charles C. Thomas.
Marshel, J. H., Kim, Y. S., Machado, T. A., Quirin, S., Benson, B., Kadmon, J., et al. (2019). Cortical layer-specific critical dynamics triggering perception. Science (New York), 365(6453), p.eaaw5202. https://doi.org/10.1126/science.aaw5202
Martens, S., & Wyble, B. (2010). The attentional blink: Past, present, and future of a blind spot in perceptual awareness. Neuroscience and Biobehavioral Reviews, 34(6), 947–957. https://doi.org/10.1016/j.neubiorev.2009.12.005
Mashour, G. A. (2014). Top-down mechanisms of anesthetic-induced unconsciousness. Frontiers in Systems Neuroscience, 8, 115. https://doi.org/10.3389/fnsys.2014.00115
Mashour, G. A., Roelfsema, P., Changeux, J.-P., & Dehaene, S. (2020). Conscious processing and the global neuronal workspace hypothesis. Neuron, 105(5), 776–798. https://doi.org/10.1016/j.neuron.2020.01.026
Mesulam, M.-M. (2000). Principles of behavioral and cognitive neurology. Oxford University Press.
Mitchell, D. B. (2006). Nonconscious priming after 17 years: Invulnerable implicit memory? Psychological Science, 17(11), 925–929. https://doi.org/10.1111/j.1467-9280.2006.01805.x
Moruzzi, G., & Magoun, H. W. (1949). Brain stem reticular formation and activation of the EEG. Electroencephalography and Clinical Neurophysiology, 1(4), 455–473. https://doi.org/10.1016/0013-4694(49)90219-9
Munk, M. H., Roelfsema, P. R., König, P., Engel, A. K., & Singer, W. (1996). Role of reticular activation in the modulation of intracortical synchronization. Science (New York), 272(5259), 271–274. https://doi.org/10.1126/science.272.5259.271
Murayama, M., & Larkum, M. E. (2009). Enhanced dendritic activity in awake rats. Proceedings of the National Academy of Sciences, 106(48), 20482–20486. https://doi.org/10.1073/pnas.0910379106
Murd, C., & Bachmann, T. (2011). Spatially localized motion aftereffect disappears faster from awareness when selectively attended to according to its direction. Vision Research, 51(10), 1157–1162. https://doi.org/10.1016/j.visres.2011.03.008
Newman, J. (1995). Thalamic contributions to attention and consciousness. Consciousness and Cognition, 4(2), 172–193. https://doi.org/10.1006/ccog.1995.1024
Pal, D., Li, D., Dean, J. G., Brito, M. A., Liu, T., Fryzel, A. M., et al. (2020). Level of consciousness is dissociable from electroencephalographic measures of cortical connectivity, slow oscillations, and complexity. The Journal of Neuroscience: The Official Journal of the Society for Neuroscience, 40(3), 605–618. https://doi.org/10.1523/JNEUROSCI.1910-19.2019
Pereira de Vasconcelos, A., & Cassel, J.-C. (2015). The nonspecific thalamus: A place in a wedding bed for making memories last? Neuroscience and Biobehavioral Reviews, 54, 175–196. https://doi.org/10.1016/j.neubiorev.2014.10.021
Phillips, W. A. (2017). Cognitive functions of intracellular mechanisms for contextual amplification. Brain and Cognition, 112, 39–53. https://doi.org/10.1016/j.bandc.2015.09.005
Phillips, W. A., Bachmann, T., & Storm, J. F. (2018). Apical function in neocortical pyramidal cells: A common pathway by which general anesthetics can affect mental state. Frontiers in Neural Circuits, 12, 50. https://doi.org/10.3389/fncir.2018.00050
Pinto, Y., Haan, E. H. F. de, & Lamme, V. A. F. (2017). The split-brain phenomenon revisited: A single conscious agent with split perception. Trends in Cognitive Sciences, 21(11), 835–851. https://doi.org/10.1016/j.tics.2017.09.003
Polack, P.-O., Friedman, J., & Golshani, P. (2013). Cellular mechanisms of brain state–dependent gain modulation in visual cortex. Nature Neuroscience, 16(9), 1331–1339. https://doi.org/10.1038/nn.3464
Powers, A. R., Mathys, C., & Corlett, P. R. (2017). Pavlovian conditioning-induced hallucinations result from overweighting of perceptual priors. Science (New York), 357(6351), 596–600. https://doi.org/10.1126/science.aan3458
Purpura, K. P., & Schiff, N. D. (1997). The thalamic intralaminar nuclei: A role in visual awareness. The Neuroscientist, 3(1), 8–15. https://doi.org/10.1177/107385849700300110
Ramon y Cajal, S. (1894). The Croonian lecture.— la fine structure des centres nerveux. Proceedings of the Royal Society of London, 55(331-335), 444–468. https://doi.org/10.1098/rspl.1894.0063
Rutiku, R., & Bachmann, T. (2017). Juxtaposing the real-time unfolding of subjective experience and ERP neuromarker dynamics. Consciousness and Cognition, 54, 3–19. https://doi.org/10.1016/j.concog.2017.05.003
Sarter, M., & Lustig, C. (2020). Forebrain cholinergic signaling: Wired and phasic, not tonic, and causing behavior. The Journal of Neuroscience: The Official Journal of the Society for Neuroscience, 40(4), 712–719. https://doi.org/10.1523/JNEUROSCI.1305-19.2019
Seifert, T., Enzinger, C., Ropele, S., Storch, M. K., & Fazekas, F. (2004). Midbrain ischemia presenting as vertical gaze palsy: Value of diffusion-weighted magnetic resonance imaging. Cerebrovascular Diseases (Basel, Switzerland), 18(1), 3–7. https://doi.org/10.1159/000078601
Sherman, S. M., & Guillery, R. W. (2001). Exploring the thalamus. Elsevier.
Steriade, M. (1981). EEG desynchronization is associated with cellular events that are prerequisites for active behavioral states. Behavioral and Brain Sciences, 4(3), 489–492. https://doi.org/10.1017/S0140525X00010037
Steriade, M., Jones, E. G., & Llinas, R. R. (1990). Thalamic oscillations and signaling. Wiley.
Steriade, M., Sakai, K., & Jouvet, M. (1984). Bulbo-thalamic neurons related to thalamocortical activation processes during paradoxical sleep. Experimental Brain Research, 54(3), 463–475. https://doi.org/10.1007/BF00235472
Suzuki, M., & Larkum, M. E. (2020). General anesthesia decouples cortical pyramidal neurons. Cell, 180(4), 666–676.e13. https://doi.org/10.1016/j.cell.2020.01.024
Takahashi, N., Ebner, C., Sigl-Glöckner, J., Moberg, S., Nierwetberg, S., & Larkum, M. E. (2020). Active dendritic currents gate descending cortical outputs in perception. Nature Neuroscience, 23(10), 1277–1285. https://doi.org/10.1038/s41593-020-0677-8
Takahashi, N., Oertner, T. G., Hegemann, P., & Larkum, M. E. (2016). Active cortical dendrites modulate perception. Science, 354(6319), 1587–1590. https://doi.org/10.1126/science.aah6066
Tasker, R. R., Organ, L. W., & Hawrylyshyn, P. (1980). Visual phenomena evoked by electrical stimulation of the human brain stem. Applied Neurophysiology, 43(3-5), 89–95. https://doi.org/10.1159/000102240
Tononi, G., Boly, M., Massimini, M., & Koch, C. (2016). Integrated information theory: From consciousness to its physical substrate. Nature Reviews. Neuroscience, 17(7), 450–461. https://doi.org/10.1038/nrn.2016.44
Tononi, G., & Edelman, G. M. (1998). Consciousness and complexity. Science (New York), 282(5395), 1846–1851. https://doi.org/10.1126/science.282.5395.1846
Tononi, G., & Koch, C. (2015). Consciousness: Here, there and everywhere? Philosophical Transactions of the Royal Society of London. Series B, Biological Sciences, 370(1668). https://doi.org/10.1098/rstb.2014.0167
Totah, N. K. B., Logothetis, N. K., & Eschenko, O. (2019). Noradrenergic ensemble-based modulation of cognition over multiple timescales. Brain Research, 1709, 50–66. https://doi.org/10.1016/j.brainres.2018.12.031
Ward, L. M. (2011). The thalamic dynamic core theory of conscious experience. Consciousness and Cognition, 20(2), 464–486. https://doi.org/10.1016/j.concog.2011.01.007
Ward, R., Danziger, S., Owen, V., & Rafal, R. (2002). Deficits in spatial coding and feature binding following damage to spatiotopic maps in the human pulvinar. Nature Neuroscience, 5(2), 99–100. https://doi.org/10.1038/nn794
Waterhouse, B. D., & Navarra, R. L. (2019). The locus coeruleus-norepinephrine system and sensory signal processing: A historical review and current perspectives. Brain Research, 1709, 1–15. https://doi.org/10.1016/j.brainres.2018.08.032
Watson, A. B. (1986). Apparent motion occurs only between similar spatial frequencies. Vision Research, 26(10), 1727–1730. https://doi.org/10.1016/0042-6989(86)90059-3
Wurtz, R. H., McAlonan, K., Cavanaugh, J., & Berman, R. A. (2011). Thalamic pathways for active vision. Trends in Cognitive Sciences, 15(4), 177–184. https://doi.org/10.1016/j.tics.2011.02.004
Xu, N.-l., Harnett, M. T., Williams, S. R., Huber, D., O’Connor, D. H., Svoboda, K., & Magee, J. C. (2012). Nonlinear dendritic integration of sensory and motor input during an active sensing task. Nature, 492(7428), 247–251. https://doi.org/10.1038/nature11601